Energy Impact Partners: A primer on the next three decades of the energy transition - Part 3
Energy Impact Partners research lead, Andy Lubershane, releases a five-part series examining technologies needed to fully decarbonize the energy system, relying on as few technology “moonshots” as possible.
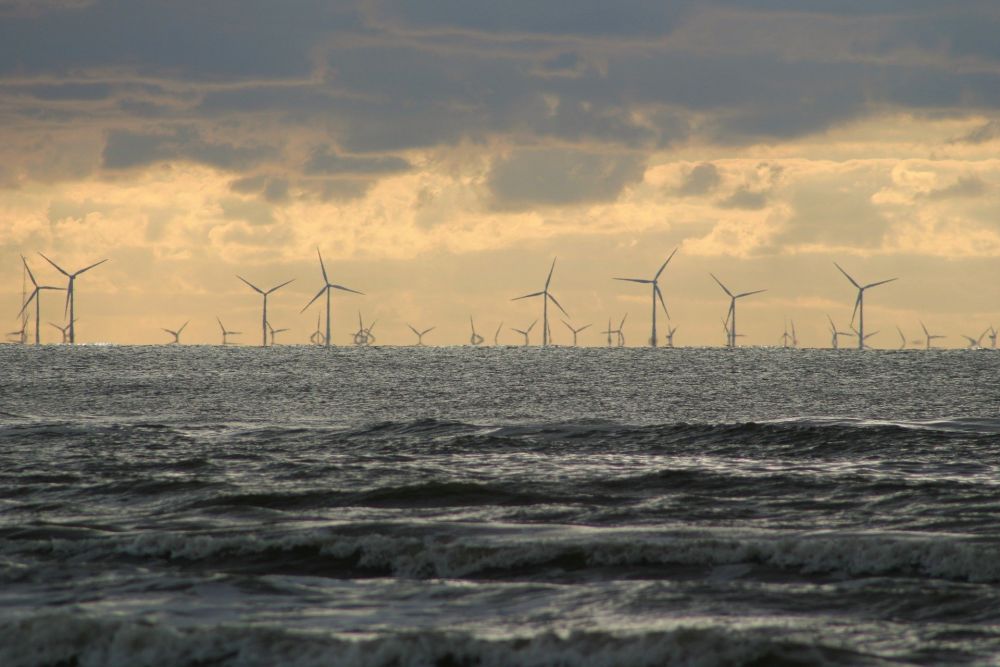
This article is the third of a five-part series examining the technology we’ll need to fully decarbonize our energy system, relying on as few technology “moonshots” as possible.
I. The Electric Future We Need
II. Two Storage Technology Revolutions
III. Cheaper, Deeper Offshore Wind: completing our renewable resource portfolio
Here’s a map of population density in the US:
Source: US Census, some awesome Reddit user
Here’s a map of wind energy resources (wind speed at 100 meters above ground):
Source: National Renewable Energy Laboratory
And lastly, here’s a map of solar resources (direct normal solar irradiance):
Source: National Renewable Energy Laboratory
These maps tell most of the story of why offshore wind needs to be a big part of our 100% clean energy mix. To make my point as plain as can be, here’s one final map, and a question: How will these roughly 100 million people get their clean energy?
The problem with us Northeasterners is that we’ve used up too much of our land already. And our land couldn’t produce a lot of energy from the wind or sun to begin with.
Moreover, we currently burn a lot of oil & gas to heat our buildings. If we were to convert all of that space heating to electricity (or to hydrogen produced via electrolysis), then our demand for electric power generation will skyrocket in the wintertime, when solar panels are at their least productive.
The density problem is somewhat unique to the Northeast region, but we’re not the only ones who’ll have a winter demand problem on our hands. Take a look at all of the states to the west of the Rocky Mountains. They have access to tremendous solar resources, especially in the Southwest, but their wind resources are pretty poor outside of a handful of breezy canyons. (Incidentally, some of those canyons in California were the site of the very first wind power installations in the country.) If these states attempt to satisfy their incremental clean energy demand through solar alone, then the amount of energy storage they need will skyrocket — both diurnally (from day to night), and seasonally.
We’re presented with two distinct problems, which I’ll refer to as a ‘northeast density’ problem, and a ‘winter nights’ problem. I’ve framed these problems within the context of the US, but you don’t have to look very hard to find versions of them in just about every corner of the world. Hence, the world needs one more clean energy resource to complement onshore wind and solar — a renewable resource that doesn’t take up much space on land, and that’s especially productive at night and in the winter.
I’m convinced that offshore wind is that resource. To understand why, let’s examine some alternative options. The best alternatives to offshore wind that might solve our Northeast density & winter night problems are:
1. A massive electric transmission expansion to deliver wind energy from the plains to the coasts
2. Three potential breakthroughs in renewable energy technology:
a. High-altitude wind turbine technology
b. Solar technology that dramatically increases photovoltaic efficiency
c. Enhanced geothermal power technology that offers a less geographically-constrained, and fully dispatchable clean energy resource
3. Options that fall into the “Savior Tech” category. (Perhaps to be discussed in an appendix…)
Let’s take these on one at a time.
Massive transmission expansion
At first blush, one possible solution to our northeast density and winter demand problems appears obvious: build more high-voltage transmission lines between the windy, sunny, open states and the calm, cloudy, crowded states! This solution has been rigorously analyzed and promoted for many years; for example, a seminal 2012 study by the National Renewable Energy Laboratory estimated that we would need to add 110–190 million megawatt-miles to our transmission grid in order to achieve 80% renewable power nationwide. Don’t worry about conceptualizing a “megawatt-mile”; what that estimate entails is roughly doubling the amount of transmission capacity we have today. Also, per NREL’s analysis, we would benefit from dramatically increasing the interconnection capacity among the three major electricity grids in the US, which today are almost entirely isolated.
China has taken a global lead in building super high-voltage, direct-current transmission lines (known as HVDC lines) across long-distances; over the past decade, these lines have been strung across China to carry renewable energy and coal generation from the remote Northwestern plains to populous, coastal cities. In the US, on the other hand, we’ve been unable to build major, inter-regional HVDC transmission lines. Why? Because the opposition of any state in the pathway of these lines can stymie a project indefinitely. In short, a big part of the problem is the phenomenon known as “not in my backyard”-ism (or NIMBY-ism, for short).
Take the Grain Belt Express, an HVDC transmission line project that was under development for many years by Clean Line Energy Partners — a venture I came to admire early on in my energy career. The proposed line would deliver wind energy from Kansas into Illinois and Indiana, and then onward into the massive mid-Atlantic energy market called the PJM Interconnection. Clean Line did the hard work of lining up wind energy sellers on one end of the Grain Belt Express, and wind energy buyers on the other. But the project has nonetheless been blocked by regulators in Missouri, which sits in the pathway of the line and stands to benefit somewhat less than its neighbors (though it would still clearly benefit).
Sadly, it’s easy to underestimate the NIMBY problem and associated political obstacles to massive transmission projects. I certainly hope that these obstacles can be overcome, but I’m doubtful they can be overcome fast enough or consistently enough for inter-regional transmission to solve the majority of our northeast density or winter night woes.
Various renewable energy technology breakthroughs:
“High-altitude” or “Airborn” wind power, means wind turbines that hover several hundred meters off the ground. (For reference, even the tallest conventional turbines today sit on top of a giant steel or concrete tower at about 120 meters.) The goal of high-altitude wind technology is to harness much stronger and more consistent wind speeds, which could hypothetically enable productivity gains of up to 50% relative to conventional turbines. The most common designs look like large kites, airplanes, or blimps that are connected to a base station via long tethers.
These base stations could hypothetically be located anywhere they can be permitted and sited, which is one of many uncertainties associated with the technology. Clearly, high-altitude wind facilities might conflict with local height restrictions and federal aviation rules. In fact, if high-altitude turbines do eventually ‘get off the ground’, coastal waters might end up being the best place to anchor them. Hence, high-altitude wind might become another form of offshore wind.
A handful of entrepreneurs have set their sights on high-altitude wind technology, but despite more than a decade of R&D efforts, none have come close to successful commercialization. Just recently, Google announced the end of its venture in the space, Makani Power. In the words of Makani’s CEO, “the road to commercialization is longer and riskier than hoped”.
Next generation solar technology may look a lot more like the current generation of solar, at least from a distance, than next-generation wind technology. Yet zooming in, the key will be to improve the efficiency of energy conversion from sunlight to electric power without increasing the cost of production. Current polycrystalline-silicon panels — mostly manufactured in Asia — are around 15–18% efficient, and have a maximum theoretical efficiency of about 32%. The most efficient panels in commercial use, made from monocrystalline-silicon, are around 22% efficient; but, they cost more to produce than polycrystalline.
The reason that panel efficiency is so important is that the benefit of efficiency ‘trickles down’ to every other component of a solar project. More energy per square meter of solar panel means more energy per acre of land (or rooftop); more energy per pound of metal in the mounting structure & concrete in the foundation; more energy per hour of installation labor. Hence, an efficiency boost of 20–30% would be a major boon to the total installed cost of massive solar farms and rooftop systems alike. Efficiency is already expected to advance incrementally, given the current trajectory of solar technology; but at this point a gain of more than a few percentage points would signify a breakthrough.
It’s important to note that this type of solar breakthrough would certainly help alleviate the northeast density problem, and reduce the overall cost of the clean energy transition, but it would do nothing to solve our winter nights problem.
Lastly, so-called “enhanced” geothermal technology has the potential to take a seat at the mainstream renewable energy table alongside wind and solar. Most of our electricity today is produced by turning heat into steam, whose lift turns a turbine. It’s very hot deep in the earth’s crust, no matter where you are on the planet — and, enticingly, that heat is available 24/7. But historically, geothermal power systems have only been cost-effective to develop where that heat has risen fairly close to the surface. Tellingly, one off the most patient, impact-focused investors in the energy tech space — Breakthrough Energy Ventures — has invested in two companies in the geothermal power space, which aim to expand the viable geographic range of the technology.
In sum: each of these technology categories is worthy of additional investment. Success in any of the three would go a long way towards solving our renewable resource shortfalls. But, they’re all challenging enough to be considered “moonshots”, or at least “moonshot-esque”. Hence, I’d prefer to bank on:
Cheaper (maybe floating) offshore wind
As recently as 2015, building wind turbines even slightly offshore, in fairly shallow water, was an extremely expensive endeavor. The levelized cost of energy for offshore wind projects, absent subsidies, was over $200 per megawatt-hour — in most locations it was the most expensive form of renewable power generation money could buy.
We have Europe to thank for footing the bill for the first generation of offshore wind projects, which were necessary in order to begin riding the learning curve towards more reasonable prices. In particular the UK, Denmark, and Germany were instrumental in pushing the technology forward. Prices have fallen by about half, to around $100 per megawatt-hour, even as projects have gradually been developed further offshore, in deeper waters. (More on this in a moment…)
One of the most visible, impactful changes in offshore wind turbine technology has been a gradual increase in the size & power generation capacity of each individual turbine. Since 2010, the size of the blades on a typical offshore turbine has increased by about 50%. Those longer blades roughly double the amount of energy that each turbine produces. On the next generation of turbines — slated to be installed in the early 2020s — each blade will be about as tall as the statue of liberty’s head.
Fortunately, it appears that much of the technological progress and manufacturing scale the industry has achieved in Europe are transferrable to the US. The first major contract signed here this decade — for the Vineyard Wind project off the coast of Massachusetts — came in at price levels comparable to those in Europe.
Source: US Department of Energy, “Offshore Wind Technologies Market Report”, 2018
Despite all of these advances, the cheapest offshore wind remains about 2–3 times as expensive as the top performing onshore wind or solar projects. Fortunately, there is plenty of room for additional progress. There are more than 25 times as many onshore wind turbines installed worldwide today as offshore turbines, and the much more well-established onshore wind supply chain is still making significant gains.
Analysis by the Department of Energy suggests that an incremental learning curve would roughly halve the cost of offshore wind energy once more, by 2030. That’s probably good enough for an economically viable solution to our northeast density and winter night problems. And yet, we could still use one more step-change in offshore wind technology, because the technology presents one more distinctive problem to contend with: a deep water problem.
So-called ‘fixed bottom’ offshore wind turbines, which are directly rooted to a foundation on the ocean floor, are currently limited to water depths of about 200 feet or less. The Department of Energy estimates that more than 60% of the total offshore wind resource in the United States is in deeper waters than fixed bottom systems are able to access. The actual percentage is likely to be higher, since waters close to shore are likely to be contested by the commercial fishing and shipping industries. Offshore projects close to shore also face the persistent threat of NIMBYism from ocean-front property owners. (The first major wind project proposed off the US coast — Cape Wind — suffered its final blow at the hands of NIMBY resistance, back in 2017.)
On the east coast, we’ll need to tap into depths of up to 350 feet. On the west coast — which has a much steeper outer continental shelf — we might be facing depths of up to 3,000 feet.
In Europe, which has deployed by far the most offshore wind of any region, project developers have not yet been forced to venture that deep; in fact, average project depth remains less than 130 feet. However, some projects are beginning to bump up against that looming 200 foot threshold. The data below illustrates that if offshore wind is going to play a major role in our energy mix, we’re probably going to need so-called “floating” turbines.
Source: US Department of Energy, “Offshore Wind Technologies Market Report”, 2018
Floating turbines still need to be anchored to the ocean floor, of course; otherwise they wouldn’t generate energy (at best they’d sail away). But, unlike the current generation of offshore turbines, they won’t be moored to the ocean floor with fixed physical structures all the way down. Instead, they’ll need to be anchored by some form of flexible mooring that can withstand the forces imposed by hundreds of feet of water, while the actual turbine structure floats on the surface.
So far, there have been a handful of experimental pilot projects to test various floating wind designs, but only one full-scale, operational project — situated off the coast of Scotland. Floating wind has quite a ways to go before it achieves commercial viability. Fortunately, we have time. In the US especially, there’s plenty of room for fixed bottom wind turbines to be installed first. Over the long-term, though, I believe cost-effective floating wind is likely to be one of the last critical pieces of the 100% decarbonization puzzle.
Speaking of 100% decarbonization: the next article in this series will focus on decarbonizing a huge category of energy consumption that can’t easily be addressed by wind power, regardless of whether it comes from on-or-offshore. That category is HEAT.